Photolysis of Organic Aerosols
A multitude of recent atmospheric observations show that organic aerosols are ubiquitous and often more abundant than other particles such as sulfate, nitrate, soot, and dust. These large amounts of organic particles contribute to the health impacts of air pollution, to regional visibility reductions, to both cooling and warming tendencies of radiative forcing, and to modified cloud properties and precipitation patterns. Uncertainties remain in all aspect of their lifecycle, i.e., their formation, transformations and properties, and especially their removal. Current chemistry-transport models assume that organic particles are removed from the atmosphere by precipitation (wet deposition)1. However, our recent modeling studies indicate that removal is dominated by other processes, specifically (a) dry deposition of organic gases followed by evaporative re-equilibration of the particles2; and (b) in the present study3 photolysis by tropospheric ultraviolet (UV) radiation.
We simulated the evolution of organic aerosols with the Generator of Explicit Chemistry and Kinetics of Organics – Atmosphere (GECKO-A) model4,5, starting with hydrocarbons typically emitted by vegetation or human activities, and computing the myriad of partly oxidized intermediate compounds including those containing chemical structures (chromophores) known to absorb UV radiation. These compounds typically have lower vapor pressures than their hydrocarbon precursors, and can partition dynamically between the gas and particle phases. Figure 1 illustrates the effect of photolysis on the concentrations of organic particles produced from the atmospheric oxidation of n-dodecane, an alkane containing 12 carbon atoms. Relative to the case where organic photolysis reactions are neglected (blue curve), inclusion of the photolysis of gas-phase species (red curve) causes a decrease in particle mass due to evaporative re-adjustment when the gas-phase species are removed by photolysis. We note that photolysis of these gas-phase organic oxidation intermediates is fairly well understood at the fundamental level, but has been viewed as too complicated for inclusion in current chemistry-transport models.
Photolysis occurring in the particle phase has been observed in some laboratory experiments using idealized systems6, but remains highly uncertain and it is unclear whether it occurs in the real atmosphere. In our simulations, if condensed organics are allowed to photolyze at the same frequency as if they were still in the gas phase, the particle mass would be reduced substantially, as shown in Fig. 1 (orange curve). An alternative estimate, based on observed UV mass absorption coefficient MAC ~ 0.1 m2 g-1 and a quantum yield of 1% for the elimination of a single carbon atom (i.e., one carbon atom ejected for every 100 UV photons absorbed), suggests an even larger reduction of organic aerosol mass (Fig. 1, black curve).
Figure 1: GECKO-A simulations of OA formation from the oxidation of dodecane (C12H26) by OH radicals.
The global impact of organic photolysis processes was examined with the GEOS-Chem global chemistry model7. The left panels of Figure 2 show the reductions in SOA mass using a quantum yield of 1% (as in Fig. 1), while the right panels show the effects of a 10% quantum yield. With the larger yields, photolysis would be the overwhelming loss process for organic particles on all scales from local to global. But even with the lower (1%) quantum yield, photolytic loss of organic particles would be of major importance, especially in aged air parcels that have experienced significant cumulative UV exposures. Notably, photolysis causes strong reductions in calculated organic particle burdens in the upper troposphere where solar radiation can be intense but rainout is irrelevant. To the extent that it occurs, photolysis modifies not only the total burden but also the geographic and vertical distributions of organic aerosols.
Figure 2: GEOS-Chem simulations of the reduction of secondary organic aerosols (SOA) by photolysis, assuming a quantum yield for the ejection of one carbon atom of 1% (left panels) or 10% (right panels).
References:
[1] Tsigaridis, K., et al., The AeroCom evaluation and intercomparison of organic aerosols in global models, Atmos. Chem. Phys., 14, 10845-10895, 2014.
[2] Hodzic, A., Aumont, B., Knote, C., Lee-Taylor, J., Madronich, S., and Tyndall, G. (2014): Volatility dependence of Henry’s law constants of condensable organics: Application to estimate depositional loss of secondary organic aerosols. Geophys. Res. Lett., 41, doi: 10.1002/2014GL060649.
[3] Hodzic et al. 2015] Hodzic, A., S. Madronich, P. S. Kasibhatla, G. Tyndall, B. Aumont, J. L. Jimenez, J. Lee-Taylor, and J. Orlando, Organic photolysis reactions in tropospheric aerosols: effect on secondary organic aerosol formation and lifetime, Atmos. Chem. Phys. Discuss., 15, 8113-8149, 2015.
[4] Aumont, B., S. Szopa, and S. Madronich (2005), Modeling the evolution of organic carbon during its gas-phase tropospheric oxidation: development of an explicit model based on a self-generating approach, Atmos. Chem. Phys., 5, 2497-2517.
[5] Lee-Taylor, J., A. Hodzic, S. Madronich, B. Aumont, M. Camredon, R. Valorso, Multiday production of condensing organic aerosol mass in urban and forest outflow, Atmos. Chem. Phys., 15, 595-615, doi:10.5194/acpd-14-17999-2014, 2015.
[6] Laskin, A., J. Laskin, and S. A. Nizkorodov, Chemistry of atmospheric brown carbon, Chem. Rev., doi:10.1021/cr5006167, 2015.
[7] Bey, I., Jacob, D.J., Yantosca, R.M., Logan, J.A., Field, B., Fiore, A.M., Li, Q., Liu, H., Mickley, L.J. and Schultz, M. (2001), Global modeling of tropospheric chemistry with assimilated meteorology: Model description and evaluation, J. Geophys. Res., 106, 23073-23096.
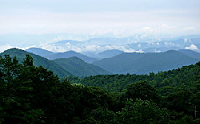